Regulatory history of non-light-water reactors in the U.S.
The Nuclear Regulatory Commission has studied issues and has written many new relevant documents to prepare for potential application submissions for non-LWRs.
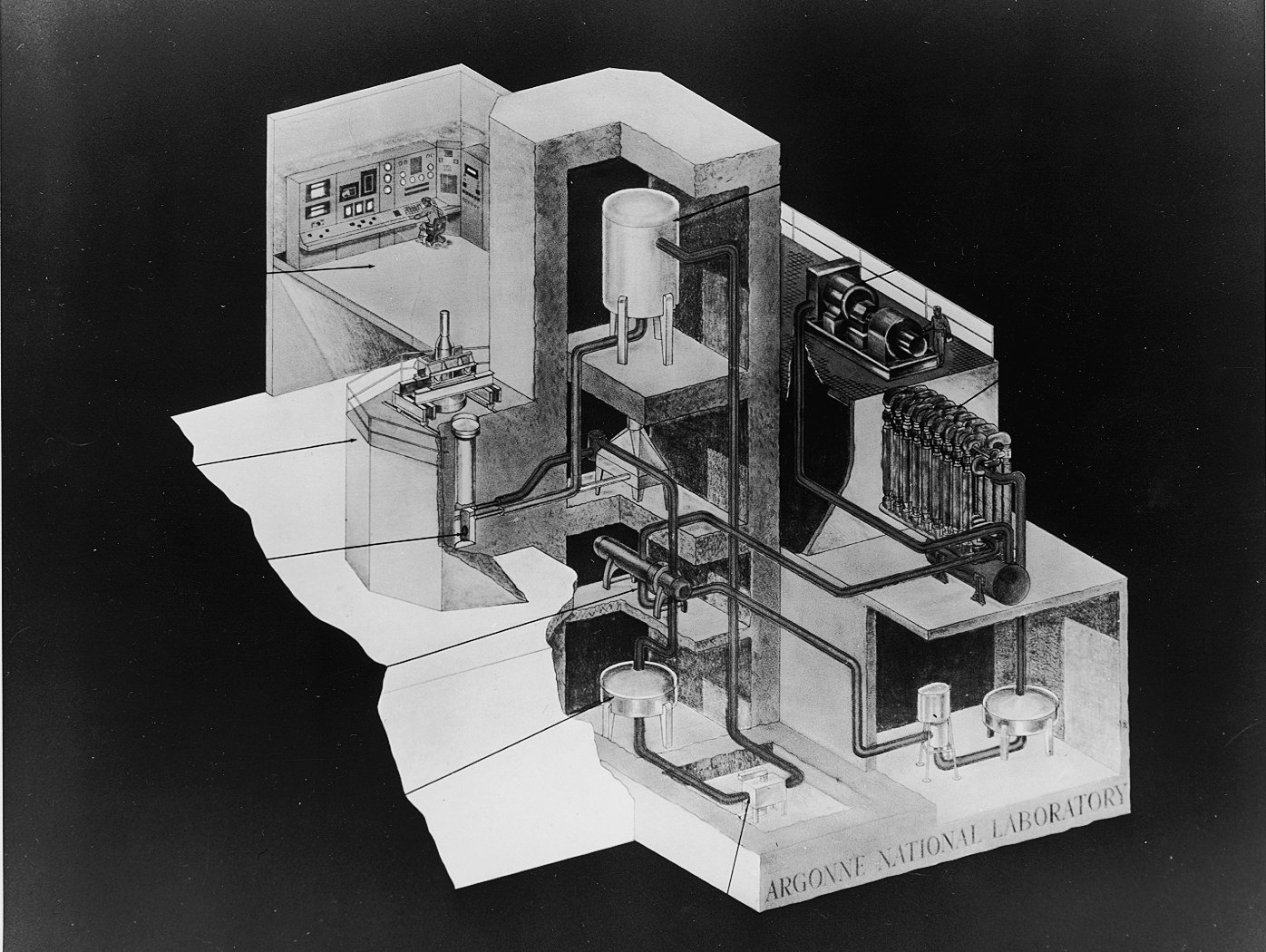
A cutaway view of EBR-1
Over the past several years there has been renewed interest in the development and licensing of advanced reactors that will be very different from the light-water reactors that are currently used to generate electricity in the United States. For example, some advanced reactors will use gas, liquid metal, or molten salt as a coolant, some will have a fast neutron spectrum, and some will be much smaller in size than current generation LWRs. The many possible applications for these reactors include electricity production, process heat, research and testing, isotope generation, and space applications.
To prepare for potential non-LWR application submittals, the U.S. Nuclear Regulatory Commission has studied the issues and written many new relevant documents. In addition, there is a long history of the NRC regulating non-LWRs that might be useful to study to help in addressing new submittals. To some extent, this has been chronicled in general histories of the NRC. Our objective herein is to describe the NRC’s history specifically with the licensing of non-LWRs and to explain some of the most salient regulatory and licensing issues.