MIT ramps 10-ton magnet up to 20 tesla in proof of concept for commercial fusion
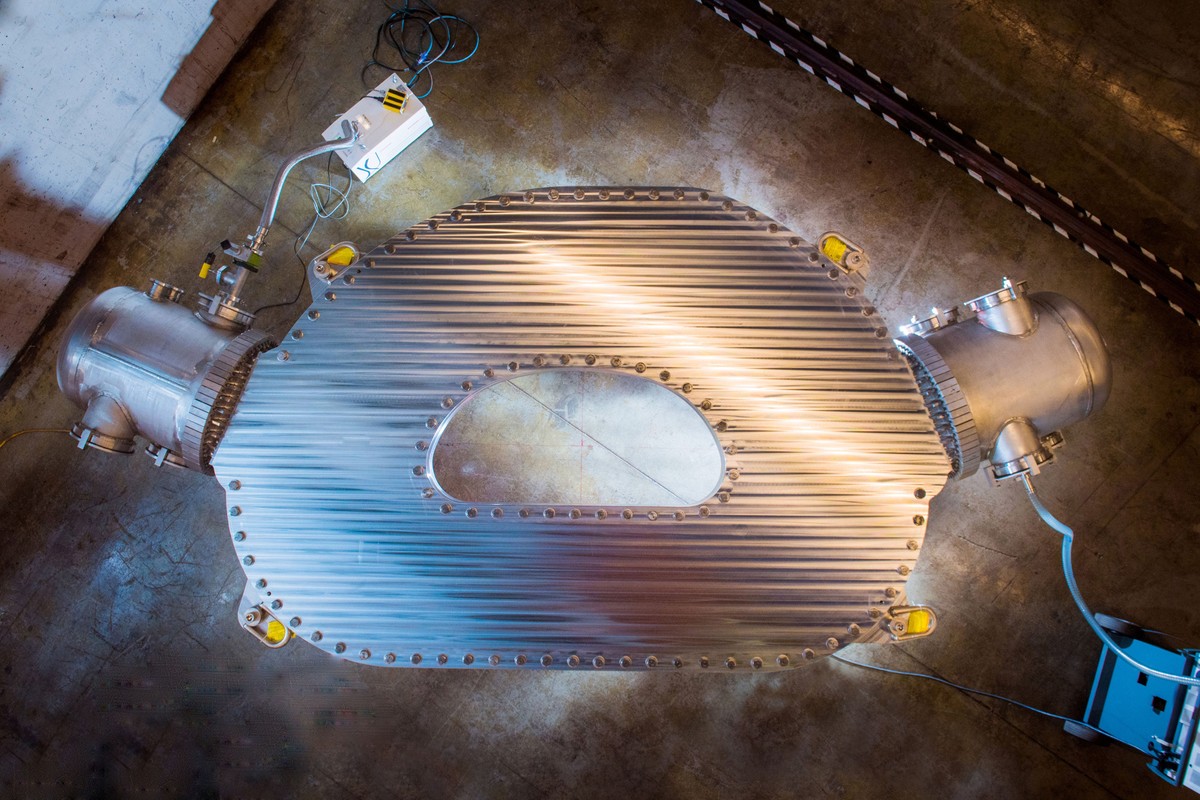
A high-temperature superconducting magnet reached and maintained a magnetic field of more than 20 tesla in steady state for about five hours on September 5 at MIT’s Plasma Science and Fusion Center. Not only is the magnet the strongest high-temperature superconducting (HTS) magnet in the world by far, it is also large enough—when assembled in a ring of 17 identical magnets and surrounding structures—to contain a plasma that MIT and Commonwealth Fusion Systems (CFS) hope will produce net energy in a compact tokamak device called SPARC in 2025, on track for commercial fusion energy in the early 2030s.